by Neil Carson Criddle
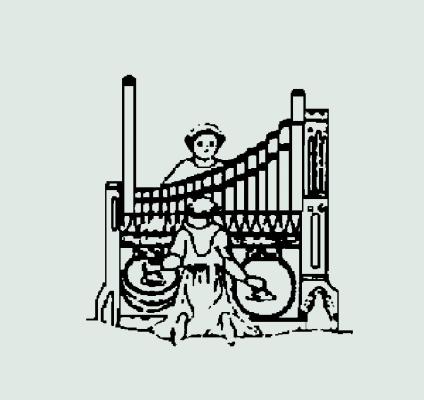
Some topics in organ building, such as history, receive much
attention. Other topics, such as turbulence in the organ wind, receive little. A major ingredient needed for turbulence ishigh speed motion with the most obvious culprit being the electric blower. Turbulence did not exist much in the days of hand-pumped bellows. It was not until electricity came along that it got a significant boost, along with wind pressure. Even so, an organ that plays with turbulence is far superior to one that sits silently due to lack of pumpers. The use of electricity has expanded since the first introduction of blowers. This has given rise to the possibility of turbulence being generated in other areas as well.
The desired air flow is nonturbulent, or laminar flow. This
happens when all the air moves smoothly in the desired direction. This is
depicted in Figure 1, which shows a wind trunk with all the air moving smoothly
in a straight path.
Turbulent flow is different. Not only does air move down the
trunk, but it also spins in large circles. The combination of these two
movements results in the air spinning in great spirals. Figure 2 is a drawing
of air spiraling down the wind trunk. Figure 3 shows the same wind trunk as
viewed from the end with the arrow depicting the circular motion.
A simple way to reduce turbulence is through the use of a
fluid collimator. (Fluid refers to any substance that flows, be it liquid or
gas.) It does this by breaking the large circular air flow into many smaller
circular flows which tend to cancel each other, thus resulting in a significant
reduction of turbulence. An easy way of making such a device is gluing pieces
of PVC pipe together and inserting it into the wind trunk. Figure 4 is an end
view of the same wind trunk showing this type of collimator and its effect on
turbulence. The large circular flows of Figure 3 cannot exist while inside the
collimator because that would require air passing through the tube walls from
one tube to another. What comes out of the collimator is many small circular
flows the same size as the tube. These smaller and numerous air flows tend to
mix and cancel each other. While there is no set rule for proportions, a pipe
length ten times greater than the diameter is reasonable. (If, for example, the
pipe diameter were 20 millimeters, the length would be 200 millimeters.)
While the collimator is very effective at reducing
turbulence, it does have a potential drawback. It is an obstacle to air flow
and thus can cause a pressure drop. The larger the pipe diameter, the less will
be the obstruction, but it will also be less effective at reducing turbulence.
Fortunately, while blowers can produce turbulence, they can also produce an
abundance of pressure. The negative side effect of obstruction is easily
overcome by simply using a higher pressure blower, if needed.
The pressure dropping effect of the collimator dictates that
it be placed upwind from the pressure sensing device, be it a pressure
regulator plate or reservoir top. It should be in the stream of unregulated
air. If placed between the regulated reservoir and the organ, there would be a
pressure drop in the organ wind. Worst of all, the pressure drop would vary
with the volume of air used. The air should also flow evenly through the entire
collimator. Beware of placing it directly in front of the blower outlet where
the air will be forced through only a small section of the collimator. If space
is a problem, it can be incorporated into the curtain valve assembly. It would
be mounted in the curtain valve plate such that the pipe ends are flush with
the plate surface. The curtain would roll up and down on the pipe ends which
support the curtain against blower pressure. However, because the air flows
only through that part exposed by the curtain, the full collimator is not taken
advantage of.
Another way to reduce turbulence is through nature. If given
time, turbulence will naturally dissipate. This can be accomplished through the
use of plenums. Plenums are simply large chambers. The reservoir is a good
example. Turbulent air enters the reservoir, mixes with other air, and has time
to dissipate. The larger the plenum, the greater this effect.
While turbulence may be produced by electric blowers, it may
also be produced by other electric devices. Electric chest action (where each
pipe has its own magnet-powered valve) has a reputation for sometimes having speech
defects during the attack. One explanation proposed for this is that the valve
opens too fast, causing the air pressure to rise too quickly. An expansion
chamber, it has been explained, between the valve and pipe, will cushion this
abrupt change in air pressure resulting in it changing more slowly, and thus
correct the problem. The problem and its correction can also be explained in
terms of turbulence. The magnet may be moving the pallet so quickly that a
region of turbulent air is created immediately above the pallet. If the pipe's
small toe hole is also immediately above the pallet, there is only turbulent
air available to enter the pipe. Instead of entering the toe hole smoothly like
stagnant air, the swirling currents of turbulent air enter the toe hole in fits
and jerks. Figure 5 shows this relationship of close proximity.
The first step in addressing this problem is to generate
less turbulence by using a slower-moving valve. This means using no more
powerful magnet than necessary. The greater the magnet's power, the faster the
pallet moves and the greater the turbulence.
The pallet can also be slowed by increasing the mass of the
armature. If weights are added to the armature, it is more massive and thus
more sluggish when it moves. Just as an automobile is more sluggish when
weighted down, so is the valve armature more sluggish when it is weighted down.
Spring tension at the other end of the armature must be adjusted so it remains
balanced. Even if there is no speech defect, the slower opening pallet eases
the pipe into speech and makes a noticeable difference in the attack. This
gentler attack is very important.
There are ways of dealing with electric chest action
turbulence after it has been created. One way is to use open toe voicing. (See
Figure 6) The larger the toe hole, the more easily turbulent air can get
through. Once through, it dissipates in the pipe foot due to the foot acting as
a plenum.
A final way to reduce electric chest action turbulence is
through the use of a plenum between the valve and pipe. The plenum provides a
space for valve turbulence to dissipate naturally just as the organ reservoir
provides a space for blower turbulence to dissipate naturally. Figure 7
provides an illustration of this.
A simple example of these corrective measures can be seen in
the cross sectional view of Figure 8. Here a plenum of large size has been
added between the valve and toeboard. In addition, weights have been attached
to the end of the armature. This increases the mass of the armature making it
more sluggish and thus slower to open. The magnet has been selected to be no
stronger than needed.
It is interesting that the oldest of organ designs, slider
chests with mechanical action, incorporates these principles. Human fingers are
very slow compared to magnets and thus insure a slow opening of the pallet. The
chest channel acts as a plenum to dissipate turbulence. Also, the modern
electric pull-down magnets in slider chests are examples of the magnets'
armatures being slowed down by the addition of mass to them. Instead of weights
glued to the armature, the large pallet acts as the mass needed to slow the
magnet.
Electric chest action has received a bad reputation. The
problem may not lie with the action but with how it is used. Electric action
provides an easy way to build a chest. Some people are attracted to it because
they do not want to put much work into building a chest. This same attitude
will carry over into other aspects of organ building such as not wanting to put
much work into voicing, planning, or any of the other innumerable areas.
Incorporating improvements such as plenums and weighting of the armature
tremendously increases the work load. Gone is the attraction of being easy.
However, in organ building it seems the one characteristic common to good
results is a lot of work. A good organ builder will come up with a good organ
regardless of what action he is given to use. The secret of success is in the
builder, not the action.
The principles of turbulence can be seen throughout the organ
from the old (slider chest with mechanical action) to the new (electric chest
action); from the microscopic (individual pipe valves) to the macroscopic
(organ blower and reservoir). The principles are everywhere, but that should
not be surprising. After all, it is all about wind.
Neil C. Criddle received his B.S. in electrical engineering
from the University of Illinois (Urbana) and the M.S. in electrical engineering
from Purdue University. While majoring in engineering he minored in music,
studying piano in college and then privately for several years, and is now
studying trumpet. He maintains an interest in the technical aspects of musical
instruments, especially the harpsichord. Criddle has built an organ in his
home, and has had articles published in the journal of the International
Society of Organbuilders (ISO).